In vitro characterisation of arterial stiffening: From the macro- to the nano-scale
- DOI
- 10.1016/j.artres.2014.01.002How to use a DOI?
- Keywords
- Material properties; In vitro mechanical testing; Arterial stiffening; Tensile testing; Nanoindentation; Atomic force microscopy; Scanning acoustic microscopy; Ageing
- Abstract
Accurate measurement of the material properties of arterial tissue is important for better characterisation of diseases and the development of reliable computational models. There are a number of in vitro techniques that are applied to study the biomechanical properties of arterial tissue. This review article presents data obtained using tensile testing, nanoindentation, scanning acoustic microscopy (SAM) and atomic force microscopy (AFM). Each of these techniques provides material property information at a different spatial resolution and in many ways are complementary techniques. The lack of consensus in the literature with regard to the appropriate stress and strain definitions that should be used when reporting tensile testing data is also highlighted. The potential of higher spatial resolution techniques, which provide data at micro-scale (nanoindentation and SAM) and nano-scale (AFM) for application to the characterisation of human aortic tissue are discussed. Finally, studies, which have examined age-related changed in the aorta at these different length scales, are highlighted.
- Copyright
- © 2014 Association for Research into Arterial Structure and Physiology. Published by Elsevier B.V. All rights reserved.
- Open Access
- This is an open access article distributed under the CC BY-NC license.
Introduction: structural basis of load transfer in arteries
Conduit arteries such as the aorta exhibit non-linear mechanical behaviour; the stiffness of arteries increases with distention. This non-linear behaviour is a protective feature preventing elastic instability such as that associated with aortic aneurysms.1 The non-linear behaviour is derived from the composite structure of the vessel wall, where elastomeric elastin acts as the compliant phase and the relatively inextensible collagen as the stiffer, reinforcing phase. It is this composite structure of the vessel wall that provides the non-linear behaviour with the initial stiffness represented by the elasticity of elastin, whereas the higher stiffness at high strains represented by fully tensed collagen fibres.1,2
The structural basis of the load transfer from elastin to collagen is the medial lamellar unit (MLU) composed of concentric elastic lamellae with smooth muscle cells and collagen fibres within the inter-lamellar spacing.3,4 As pressure increases, there is a progressive straightening of the lamellae with a corresponding decrease in the inter-lamellar distances.1 This circumferential straightening of elastin layers and the alignment of collagen fibres with distention under physiological pressures correlates with the increasing elastic modulus observed by mechanical testing in vitro.1
Experimental observations with electron microscopy have led to the refinement of the original MLU model.5,6 It is now clear that the MLU is highly complex and that the arrangement of the main constituents correlates with the known mechanical behaviour of the aorta. The three primary medial constituents (collagen bundles, smooth muscle cell nuclei and inter-lamellar elastin fibres) are all found to be predominantly orientated in the circumferential direction corresponding with the circumferentially dominant values of physiological stress.6
Hence, given that arteries have a multi-layered anatomical structure and a complicated organisation of cellular and extracellular matrix components within these layers, it would be expected that with ageing and vascular diseases associated with increased arterial stiffening, that there is mechanical degradation within arteries at the macroscopic level (encompassing the adventitia, media and intima), microscopic (within a specific anatomical layer or structural unit such as the MLU) or at the molecular or cellular level.7 Similar to findings for cartilage,8 it may be that decline in arterial structure begins at the molecular level and progresses to the higher levels of architecture. Hence, there is a need to determine localised mechanical properties for vascular tissue and relate these properties to its macroscopic structure and function. This will enable better characterisation of changes that occur due to the vasculature with ageing and disease, the development of reliable numerical models, and ultimately improved clinical treatments.
This review article will focus on a number of in vitro techniques that can be used determine the biomechanical properties of arteries at different length scales (macro- to nano-scale), and highlight data reported in the literature which considers age-related changes in arterial stiffening across these length scales.
Macromechanical testing
Uniaxial tensile tests where rectangular specimens are cut from vascular tissue, mechanically clamped and subjected to an axial tensile force (Fig. 1) yield a similar shaped stress–strain curve as an incremental elastic modulus-wall stress curve (which are widely used to characterise in vivo biomechanical properties of the arteries) e.g.9,10 Tensile testing is a useful technique for biomechanical characterization of vascular tissues as a number of parameters can be extracted from the resulting stress–strain curves.11
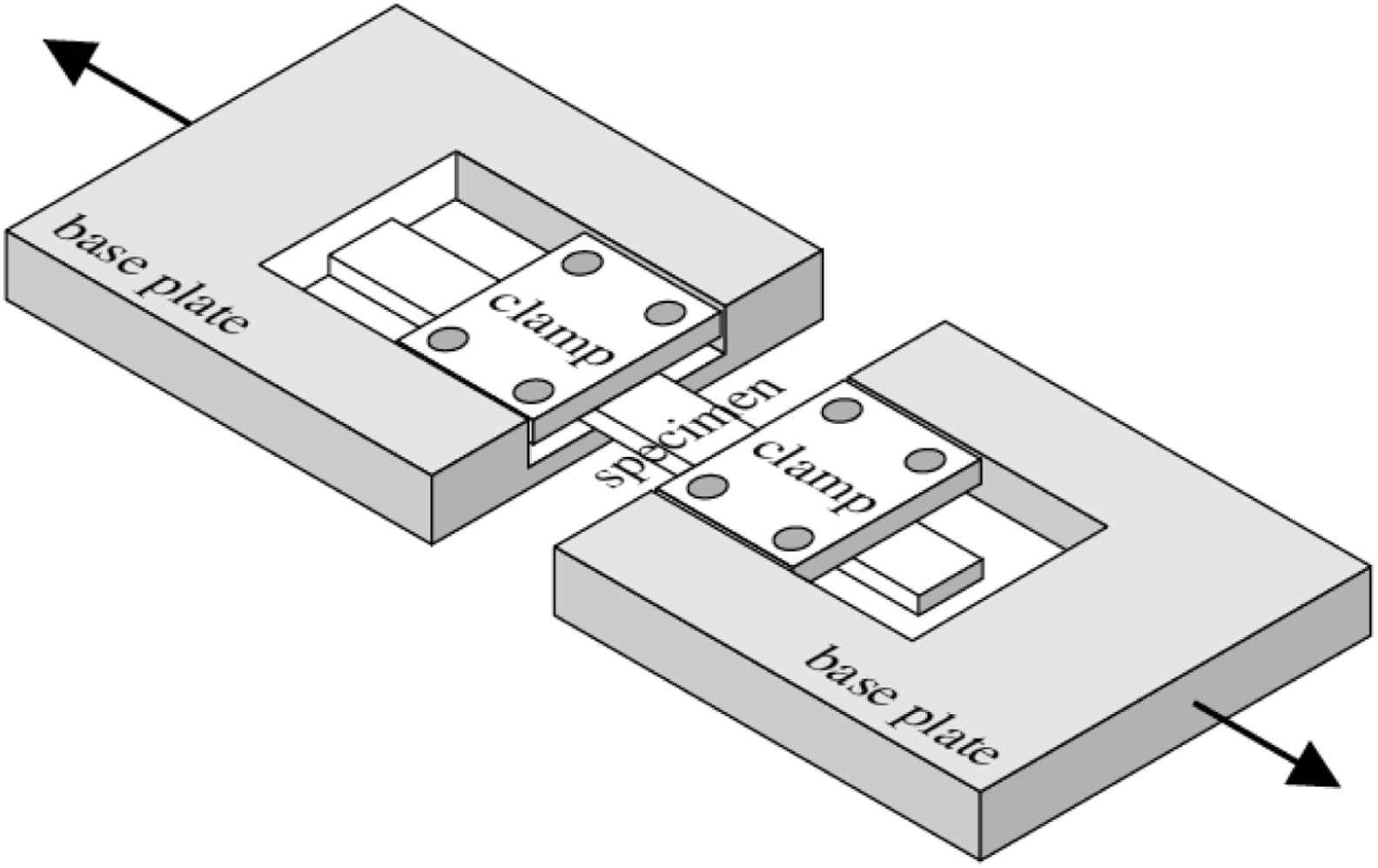
Schematic showing sample setup for an uniaxial tensile test. A rectangular section of tissue is prepared and clamped as shown. Reproduced from Ref. 12 with permission from Highwire Press.
The mechanical properties of aortic aneurysm as compared to non-aneurysmal aorta have been determined in a number of studies using uniaxial tensile testing e.g.13,14 The tensile strength of ascending thoracic aortic aneurysm tissue is found to be 29% and 34% less than that of control tissue in the longitudinal and circumferential orientations respectively.14
One of the issues with the stress–strain behaviour reported in the literature for aortic aneurysm tissue using tensile testing is the definition of stress and strain.11,15 The most commonly used definitions for stress and strain are engineering stress and engineering strain.16 Here, it is assumed that stress (σE) is uniformly distributed over the cross-sectional area of the sample and is equal in magnitude to
Similarly, engineering strain (εT) is assumed to be constant over the sample gauge length (l) and equal to
Although these definitions may be true in some engineering applications where the cross-sectional area and length of the specimen do not change substantially during loading, the relationships do not hold true for biological tissues where the cross-sectional area and the length of the specimen change substantially during testing. In such cases it is more appropriate to use alternative measures of stress and strain. True stress (σT) is defined as the ratio of the applied load to the instantaneous cross-sectional area (A) of the specimen. True stress can be related to the engineering stress by assuming there is no change in volume:
Hence,
True strain is defined as the sum of all the instantaneous engineering strains.
True strain (ε↓T) is therefore related to engineering strain as follows
As noted by Duprey et al.11 and Khanafer et al.,15 some studies on aortic aneurysm tissue report engineering stress versus engineering strain whereas others report true stress versus engineering strain, although the rationale behind this latter is not clear. Other definitions of stress and strain are also utilised for the study of aorta mechanics. Khanafer et al.15 present a critical comparison of the various definitions of stress and strain as applied to aortic tissue and the implications for the resulting material response. Table 1, based on the work of Khanafer et al.15 summarises some of the different studies on human aortic tissue with tensile testing.
Reference | Tissue | Testing method | Sample geometry | Stress | Strain |
---|---|---|---|---|---|
He and Roach17 | Abdominal aortic tissue | Uniaxial | Rectangular strips | True | Engineering |
Vorp et al.14 | Ascending thoracic aorta | Uniaxial | Rectangular strips | True | Engineering |
Iliopoulos et al.18 | Thoracic aortic tissue | Uniaxial | Rectangular strips | True | Engineering |
Raghavan et al.13 | Abdominal aortic tissue | Uniaxial | Rectangular strips | True | Engineering |
Duprey et al.11 | Ascending thoracic aorta | Uniaxial | Rectangular strips | True | True |
Choudhury et al.19 | Ascending aortic tissue | Biaxial | Square sections | Engineering | Engineering |
Vande Geest et al.20 | Abdominal aortic tissue | Biaxial | Square sections | Second Piola–Kirchhoff | Green strain |
Summary of some of the tensile testing methods and stress–strain definitions used in the literature for the study of aneurysms on human tissue. Definitions of Second Piola–Kirchhoff and Green Strain are provided in Ref. 15.
As shown in Fig. 2, their study demonstrates that the maximum elastic modulus can be much higher than when the true stress is used rather than the engineering stress. They conclude that the engineering stress–strain curve is not suitable for aortic tissue testing because it does not give a true indication of the tissue’s deformation characteristics.
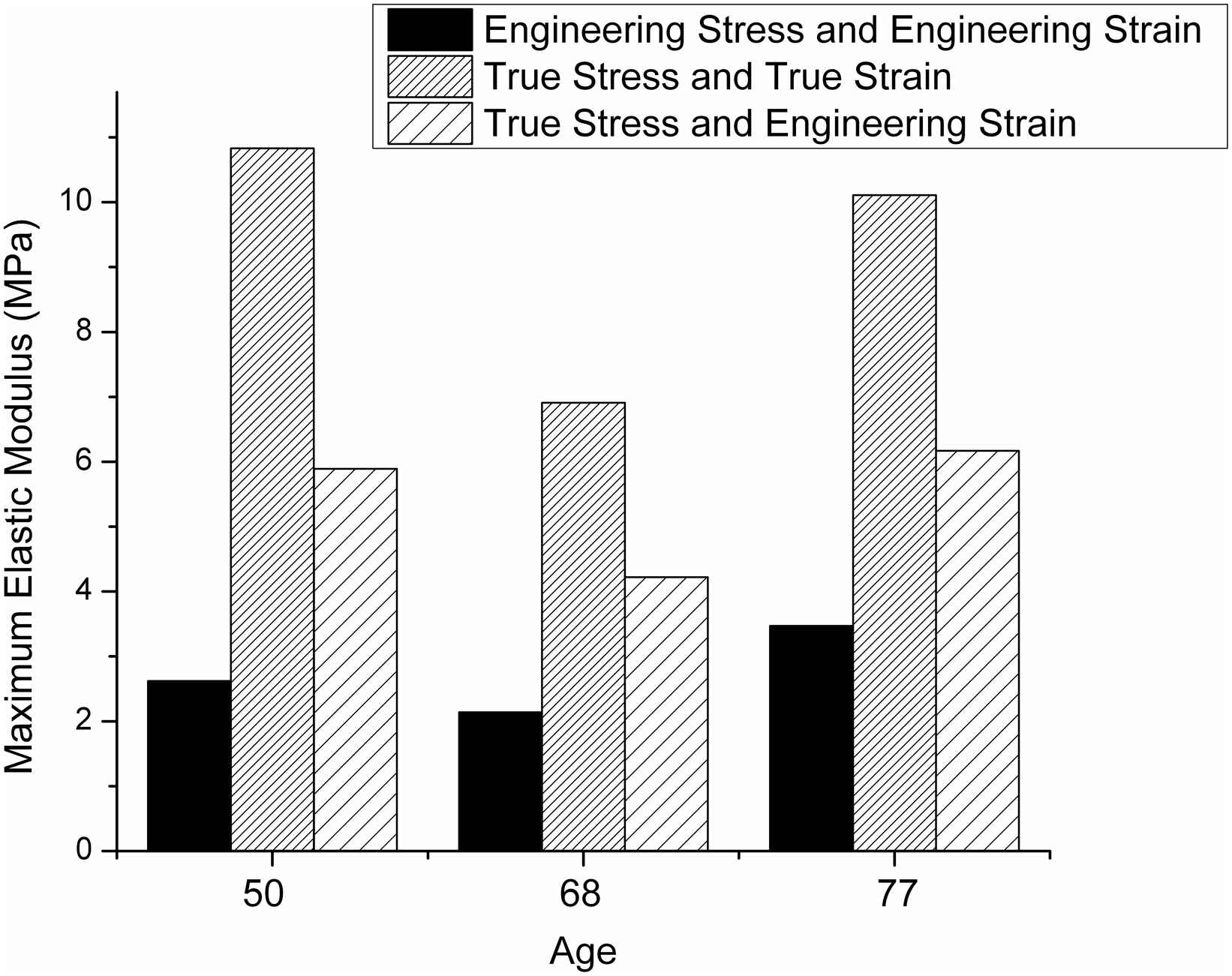
Bar graph based on data reported by Khanafer et al.15 showing variation of the maximum elastic modulus values (determined from the upper region of the stress–strain curve) for various stress–strain definitions. The data were obtained using a circumferential specimen from patients with ascending thoracic aortic aneurysm.
It should also be noted that Young’s modulus is a suitable measure of stiffness for linearly elastic engineering materials where the Young’s modulus is a constant. As stated earlier, arteries exhibit hyperelastic behaviour i.e. their stiffness increases with an increase in stress and strain. Hence, tangent modulus rather than Young’s modulus is a more appropriate measure of stiffness. Tangent modulus refers to the slope at a given point on the non-linear stress–strain curve and is generally reported for a given value of stress.21
Aside from human aorta samples, tensile testing has also been used to characterize human coronary arteries. Claes et al.22 found that the circumferential tensile strength of human coronary arteries decreases from approximately 1.5 to 0.5 MPa with age (utilizing 5 samples with the age range covering 23–83 years). Holzapfel et al.23 have conducted tensile testing on isolated intima, media and adventitia layers from human coronary arteries and found that the intima showed load-bearing capacity and mechanical strength as compared with the media and adventitia. This study is unique because it is one of the few biomechanical studies using tensile testing which does not consider the arterial wall to be a single homogenous layer.24 However, the structural integrity of the tissue is compromised by separating the arterial layers in this manner.
Tensile testing has also been used widely to characterize the in vitro biomechanical properties of animal vascular tissue e.g.25–31 Detailed coverage of these studies is beyond the scope of this paper.
Micromechanical testing
In recent years, there has been an interest in applying nanoindentation to characterise the biomechanical properties of the aorta. Nanoindentation is used widely for characterization of engineering materials. With nanoindentation, a rigid indenter, usually constructed of diamond, is used to probe small volumes of a material. The load and displacement of the indenter are recorded and these are used to measure the elastic modulus and hardness of the material. A typical nanoindentation load–displacement curve is shown in Fig. 3. The technique is used extensively for determining localized mechanical properties of mineralized tissues but more recently it has been applied to the characterization of soft tissues.32
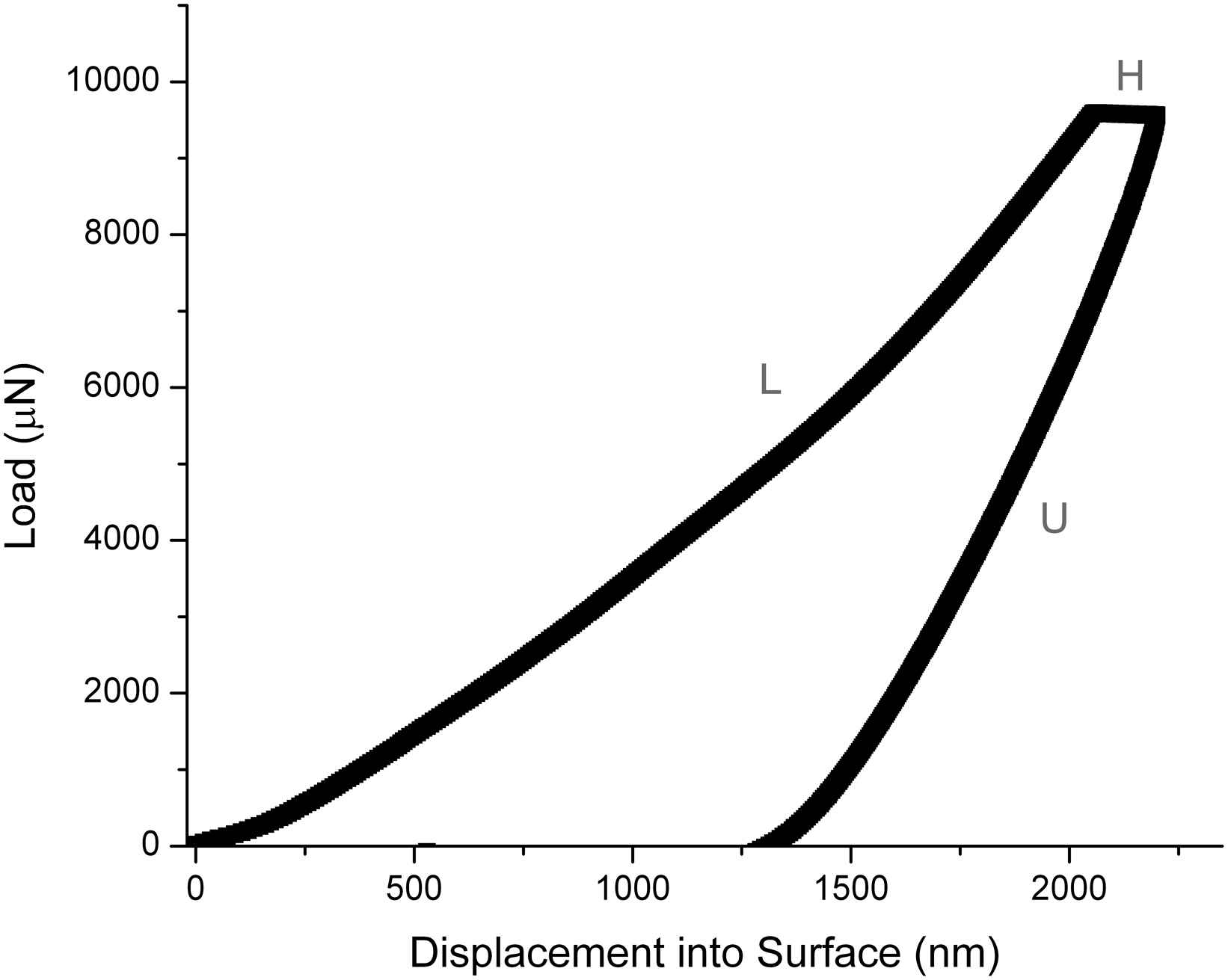
Typical nanoindentation load–displacement curve. A typical test involves a load (L), hold (H) and unload (U) region. During the hold period, biological materials show an increase in displacement whilst the load is help constant because they exhibit creep behaviour (time-dependent deformation).
Nanoindentation has several advantages over traditional mechanical testing techniques such as tensile testing due to its ability to characterise very small, heterogeneous samples. Hence, it has potential application for characterising tissue from biopsy samples and tissue from small animal models.32 However, testing of soft tissues such as the aorta is much more challenging (as compared to mineralised tissues) with commercial nanoindentation systems due to the high surface roughness and low stiffness of such samples.
A number of approaches have been utilized to overcome the technical difficulties associated with testing of soft tissues. For stiff materials, which have an elastic modulus on the order of gigapascals, it is possible to determine the properties of the sample with sharp indenter tips such as a Berkovich tip (a three-sided pyramid), which typically have a radius of curvature <200 nm. However, for soft tissues a trade-off is made with spatial resolution to obtain reproducible results by using larger radius tips with either flat or rounded ends. Ebenstein and Pruitt33 utilized 100 μm radius conospherical indenters to determine the elastic properties of agarose and gelatin gels with a view of developing a method suitable for application for vascular tissues. The technique was applied to characterise hydrated, porcine aortic tissue. With the technique, the aorta was found to exhibit similar mechanical behaviour as the 5% agarose gel. Levental et al.34 developed a custom indentation device with a 255 μm probe and determined the storage modulus of mouse aorta sections as a function of the distance from the heart. The storage modulus (G′) represents the stored energy in viscoelastic materials i.e. elastic behaviour. They reported that the aortic arch had the lowest storage modulus (G′ = 82 ± 44 Pa) followed by the abdominal aorta (169 ± 69 Pa) and the thoracic aorta (220 ± 93 Pa). The use of such a relatively large indenter tip provides the average mechanical properties of the mouse aorta rather than the properties of individual components or regions of the tissue.7,32
Other studies have attempted to use nanoindentation to differentiate the local mechanical properties of the distinct anatomical layers of the aorta. Hemmasizadeh and co-workers24 were able to successfully apply nanoindentation to porcine aorta samples with a 10 μm conospherical tip with the aim of differentiating between the different layers of the aortic wall. The smaller radius probe allowed the elastic and viscoelastic properties of the ‘outer’ and ‘inner’ layers of the aorta (corresponding to the adventitial and medial regions of the aortic wall respectively) They found that the outer adventitial layer was stiffer and showed less relaxation, as determined with the Quasi-linear Viscoelastic (QLV) model.35 These data agree with the trend reported in our earlier study36 conducted on histological sections of ferret aorta, also with a 10 μm conospherical tip. In our study, we reported that the elastic modulus decreased progressively from the adventitial to the intimal layer of the vessel wall. We related the elastic modulus to the distribution of elastic fibres within the vessel wall using fluorescence microscopy. The elastic modulus was found to inversely correlate with elastic fibre density.36
Nanoindentation may develop as a powerful tool to better characterise and diagnose pathological changes in large arteries. However, to date there has been little application of the technique to clinical problems. The technique has been used to characterise human atherosclerotic plaque tissue.37 Nanoindentation is ideal for such characterisation due to the heterogeneity of plaque tissue. Ultimately, nanoindentation may not only assist the development of more accurate computational models for atherosclerosis plaques but may also aid in better characterisation and enhanced treatment of vulnerable plaques.37
Another engineering technique which can be used for determining mechanical properties of vascular tissue is scanning acoustic microscopy (SAM). SAM measures the elastic propagation of acoustic waves (typically with a frequency of 100 MHz–1 GHz) through a material. One of the advantages of SAM over other techniques is that it is an imaging technique and without any staining or special preparation methods, the contrast in the acoustic image is sufficient to resolve distinct features hence SAM can provide both histological and mechanical information7 (Fig. 4).
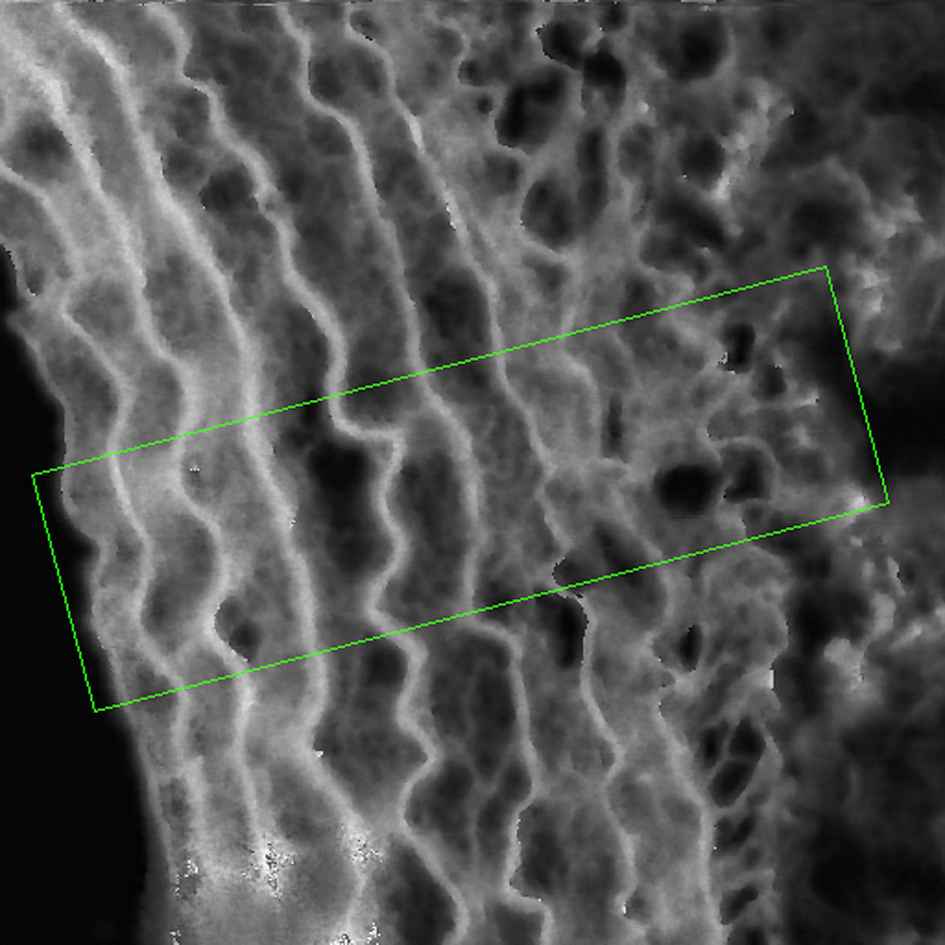
Typical processed SAM image of a rat aorta section obtained using our Multi-Layer Phase Analysis Method (MLPA).38 Higher acoustic wave speeds are represented by the brighter pixels. Image size is 200 μm × 200 μm. Specific regions of interest can be selected to extract acoustic wave speed values off-line. A region of interest is highlighted with the rectangular box profile. The adventitial surface of the image is on the right hand side of the image.
At 1 GHz, the spatial resolution of the technique is around 1 μm. The acoustic wave speed (c) is related to tissue stiffness as follows:
We have used SAM to examine age-related changes in an ovine model of ageing and found that firstly, acoustic wave speed increases with age, and secondly that the increased acoustic wave speed in the aged aorta can be localised to the inter-lamellar region of the MLU.39 In a later study with SAM we examined the role of a cytokine, Cardiotrophin 1 (CT-1), on vascular stiffening in a rat model.10 Here, we reported an increase in acoustic wave speed in the CT-1 treated rat aortae, matching in vivo mechanical property measurements. The greatest increase in acoustic wave speed was also localised to the inter-lamellar region in this study.
Nanoindentation and SAM are complementary techniques and can be combined to determine two independent elastic constants for a sample. By combining both techniques, the Poisson’s ratio and elastic modulus can be accurately determined without prior knowledge of either. This is because the mathematical relationship between Young’s modulus and Poisson’s ratio is different for the two experimental techniques.40
Nanoscale mechanical testing
At the nanoscale, atomic force microscopy (AFM) can be used to determine localised mechanical properties of biological tissues. AFM utilises a sharp probe (typically < 10 nm) mounted on a compliant cantilever. When the tip and sample are brought into contact, forces between the tip and sample lead to a deflection of the cantilever. The deflection is recorded by a laser light that is reflected from the top surface of the cantilever on to a photodetector. Hence, unlike a nanoindenter, where an external load is applied to an indenter tip, with AFM the elastic deflection of the cantilever is monitored to measure the forces acting on the probe.41 A comparison of typical hardware found in commercial AFM and nanoindenter instruments is presented in Ref. 41. Sherratt42 compares and contrasts the techniques when applied to biological tissues in terms of sampling frequency and resolution.
Although absolute mechanical property data obtained with AFM can be considered semi-quantitative due to inherent limitations of the technique,43 AFM is an appealing and versatile technique as it provides high spatial resolution images of tissue ultrastructure such as individual collagen fibrils44 and can complement electron microscopy imaging of aortic ultrastructure.45 This section will highlight those studies where AFM has been used for indentation measurements.
The high spatial resolution of AFM readily allows mechanical properties of distinct regions of arteries to be determined. Peloguin et al.46 were able to characterise subendothelium mechanics in bovine carotid arteries using AFM-indentation. AFM-indentation is used widely to characterise cell mechanics and in studies of vascular mechanics has been used to characterise rabbit abdominal aorta endothelial cells,47 and pulmonary artery smooth muscle cells obtained from patients with idiopathic pulmonary arterial hypertension.48
AFM-indentation has been used to measure nanoscale changes in the mechanical properties of porcine aorta following selective removal of different extracellular components.49 In this study, the authors’ found that although the removal of collagen led to a ∼45-fold decrease in adventitia and ∼3 decrease in the media, the remaining tissue still formed a coherent network.
Grant et al.50 have recently used an AFM-based indentation technique to determine the nanomechanical and viscoelastic properties of the tunica adventitia of the porcine aorta and pulmonary artery. They demonstrated that the aortic adventitia exhibits greater time-dependent behaviour than the pulmonary artery. The behaviour fits with the differing cardiovascular function of the two vessels.
In a scaled up version of an AFM instrument, Matsumoto et al.51 examined the mechanical properties of lamellar and inter-lamellar regions (which they denote a smooth muscle-rich layer) separately in porcine thoracic aortae. With this custom instrument, the scanning micro-indentation tester (SMIT), they found that the lamellar regions were 2.5 stiffer than the inter-lamellar regions of the aorta. Our SAM studies10,39 also resulted in higher acoustic wave speed values in the lamellar regions relative to inter-lamellar regions but we did not find such a substantial difference.
Tensile testing versus indentation
Currently, there has currently been little comparison in the literature with regards to the differences in the values of Young’s modulus that are reported with indentation measurements (such as nanoindentation and AFM) as compared to tensile tests. The modulus for a number of soft tissues appears to be consistently lower when determined with indentation techniques as compared to tensile testing.52
All of the in vitro mechanical property measurement techniques that have been covered in this review are complementary although the absolute values obtained with these techniques are not directly comparable due to the different spatial resolutions of the techniques. This is discussed further in Ref. 7.
Ageing arterial tissue: from pulse wave velocity to cell stiffness
A detailed summary of age-related changed in elastic arteries is presented by O’Rourke and Hasimoto53 and Greenwald.54 With age, these arteries dilate and become stiffer. Individual elastin lamellae become thinner and separated by increasing amounts of nonload-bearing material. This is shown schematically in Fig. 5.
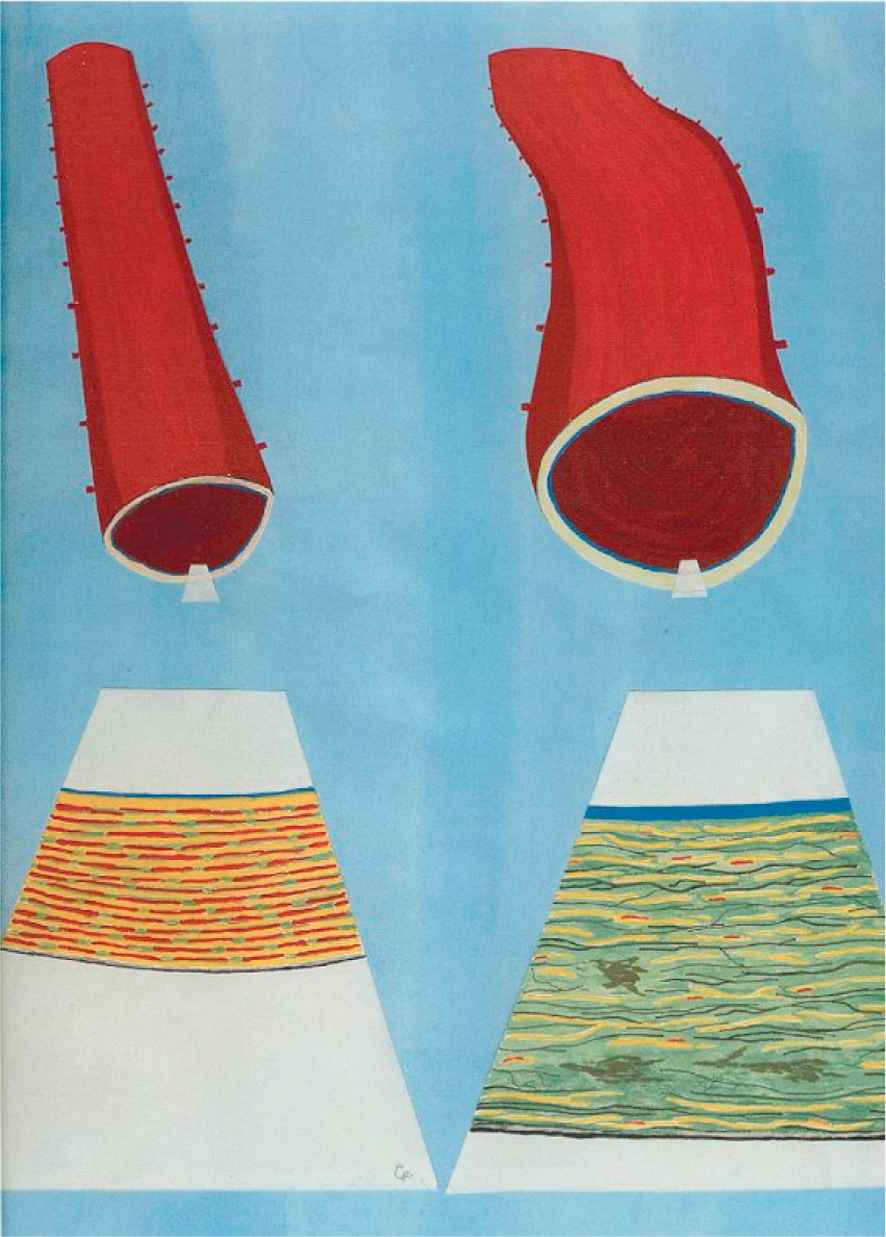
Schematic diagram representing age-related changes in the human aorta with the left and right images representing a young and old aorta section respectively. In the aged aorta, the microstructure is more disorganised due to fraying and fracture of the elastic lamellae (shown in yellow) and loss of muscle attachments (shown in red). There is also an increase in collagen fibres (black) and mucoid material (green), and with foci of medionecrosis.55 Reproduced from Ref. 53 with permission from Elsevier. (For interpretation of the references to colour in this figure legend, the reader is referred to the web version of this article.)
O’Rourke and Hasimoto53 state that these physical phenomena can be understood by considering the engineering principles of fatigue and fracture, in particular in relation to ageing. Within the aorta, fracture of elastin is seen with age.56 This can explain dilation, because there is a fracture of load-bearing material, and stiffness, because stress is transferred to the stiffer collagen components of the arterial wall.53
In vivo aortic stiffness data collected with carotid-femoral pulse wave velocity (PWV) demonstrates that PWV increases from approximately 7 ms−1 at ages <30 to around 11 ms−1 at ages ≥70 for normal levels of blood pressure.57 The clinical perspective and current understanding of arterial stiffness in the context of ageing is reviewed by Cavalcante.58
At the macroscopic level, a comprehensive in vitro biomechanical study by Okamoto et al.59 was conducted on dilated human aorta samples using biaxial mechanical testing. Their data show that mean strength decreases from 2.04±0.46 MPa in tissue from younger patients (characterised as <50 years) to 1.35 ± 0.37 MPa. Vande Geest et al.,60 also with biaxial tensile testing, characterised age-related changes in human infrarenal abdominal aortic tissue. They found that the stress–strain response changes from a sigmoidal shape of high extensibility for young tissue to a much stiffer exponential shape displaying a significantly lower areal strain for older tissue (areal strain is the change in area between the reference and deformed states of the tissue).
There are currently no published studies at the micromechanical level on age-related studies on human aortic tissue. However, SAM has been used to study human aortic tissue previously. Saijo et al.61 have characterised atherosclerotic human aorta samples with SAM and also aortic aneurysms.62 Our work to date with SAM has mainly focussed on animal models. Our data, which shows that age-related stiffening is localised to the inter-lamellar regions of the MLU, was presented earlier. There is plenty of scope for the micromechanical techniques that have been highlighted in this paper to be applied to ageing studies on human aortic samples.
Using aorta from young and old male monkeys, Qui et al.63 examined the elastic modulus of vascular smooth muscle cells with AFM-based indentation. They report that the apparent elastic modulus increased from 12.8 ± 0.3 kPa to 41.7 ± 0.5 kPa. To our knowledge, this is the only nanoscale study of ageing within a component of the aortic wall using AFM-indentation. We are currently using AFM to investigate the morphology and mechanical function of fibrillin microfibrils isolated from rat aorta in an experimental model of diabetes.64 Although this work does not involve indentation, we are able to investigate the tensile strength of the microfibrils using molecular combing.65 Fibrillin microfibrils have an important role in the elasticity of the aorta65 and these data will shed light on the mechanical properties of a key component of the aortic wall at the nanoscale. There is considerable scope to apply these techniques to examine age-related changes in the aorta.
Summary
In vitro mechanical testing techniques are powerful characterisation tools to assess changes in arterial biomechanical function. Accurate measurements from such techniques are vital for the development of reliable numerical models and improved clinical treatments. Uniaxial tensile testing is used widely for biomechanical characterisation of aortic aneurysms, however a number of different stress and strain definitions are used in the literature. Techniques such as nanoindentation, SAM and AFM, which can probe higher spatial resolutions than conventional mechanical testing methods, have significant potential for assessment of arterial stiffness. In animal models of ageing, there is data at the micromechanical and nanomechanical level. There is much scope to apply these to human arterial tissue.
Acknowledgements
I am grateful to Professors Brian Derby (University of Manchester) and Kennedy Cruickshank (King’s College, London), and Dr Mike Sherratt (University of Manchester) who have contributed greatly with their materials science, clinical and biochemical expertise respectively to our studies. Thanks are due to Drs Natalie Gardiner and Xuegen Zhao (both at University of Manchester) who provided the aorta tissue and conducted the SAM imaging respectively (shown in Fig. 4).
References
Cite this article
TY - JOUR AU - Riaz Akhtar PY - 2014 DA - 2014/01/26 TI - In vitro characterisation of arterial stiffening: From the macro- to the nano-scale JO - Artery Research SP - 1 EP - 8 VL - 8 IS - 1 SN - 1876-4401 UR - https://doi.org/10.1016/j.artres.2014.01.002 DO - 10.1016/j.artres.2014.01.002 ID - Akhtar2014 ER -