Structural proteins and arterial ageing
- DOI
- 10.1016/j.artres.2012.12.001How to use a DOI?
- Keywords
- Arteriosclerosis; Elasticity; Atomic force microscopy; Nanoindentation; Oxidative damage
- Abstract
In common with most dynamic tissues, the mechanical properties of arteries are determined by the relative composition and architecture of key structural extracellular matrix (ECM) proteins including fibrillar collagens and elastic fibre components. Although it is apparent that age-related loss of ECM homeostasis leads to arteriosclerosis (vascular stiffening), which in turn is associated with the development of both fatal strokes and heart failure, the principal molecular targets and causative mechanisms remain poorly defined. This review discusses: (i) the application of in situ micro-mechanical methodologies to localise the key molecular targets of age-related stiffening within arteries and other dynamic elastic tissues and (ii) the potential role played by the preferential oxidation of TGF-β binding elastic fibre-associated components in driving this aberrant tissue remodelling.
- Copyright
- © 2012 Association for Research into Arterial Structure and Physiology. Published by Elsevier B.V. All rights reserved.
- Open Access
- This is an open access article distributed under the CC BY-NC license.
Introduction: clinical and structural consequences of arterial ageing
The mechanical properties of dynamic connective tissues such as arteries are mediated by both intracellular and extracellular structural proteins. Intracellularly, there is evidence that the actin cytoskeleton influences the stiffness of vascular smooth muscle cells (VSMC) and hence the vessel1 whilst in the extracellular space fibrillar collagens resist compressive and tensile forces respectively2 and elastic fibres confer compliance and passive recoil.3 However, pathological changes to these mechanical properties are a common feature in ageing mammalian tissues including skin,4 lungs5 and arteries.6,7 In the specific case of large arteries diffuse stiffening (termed arteriosclerosis7,8) is implicated in the maintenance of essential hypertension9 and, in turn, with the development of stroke and heart failure.10,11 Unfortunately the key causes of arteriosclerosis remain poorly defined7 and this lack of consensus is due, in part, to the complexity of the structural and functional remodelling which accompanies ageing (Fig. 1A).
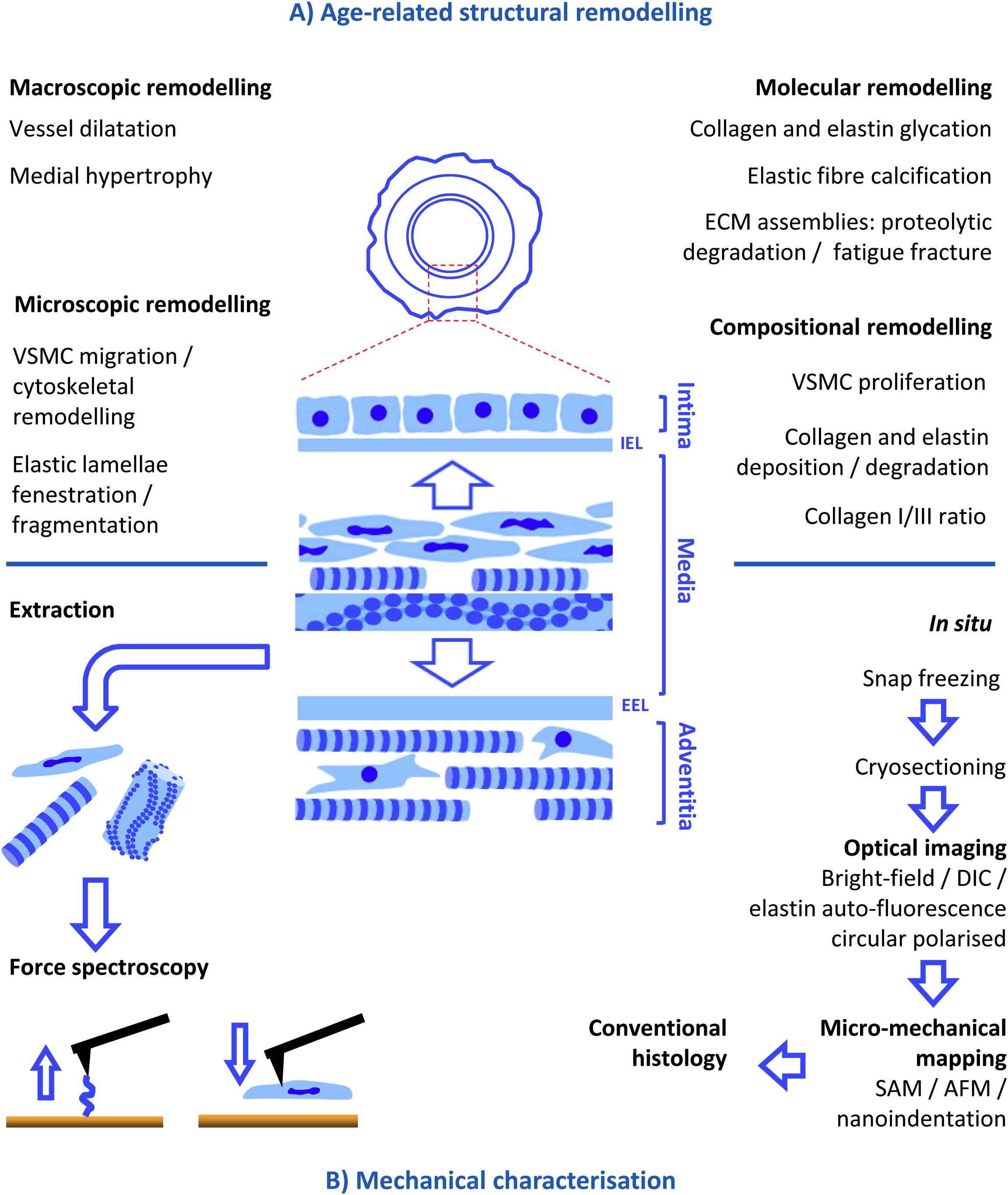
Structural remodelling in, and mechanical characterisation of, ageing arteries. Large arteries such as the aorta are composed of three layers: (i) an inner, endothelial cell-containing, intimal layer, (ii) a medial layer which is characterised by concentric medial lamellar units (composed of elastic lamellae and collagen and VSMC-rich interlamellar regions) and is demarcated from the other layers by an inner and outer elastic lamellae (IEL and EEL) and (iii) an outer, collagen-rich, advential layer. (A) This complex structure undergoes equally complex remodelling at macroscopic to molecular length scales during ageing (see text for references) which in turn induce age-related stiffening. (B) The cause(s) of arterial stiffening can be clarified by localising mechanical changes to specific components either by extraction (and subsequent testing of the extracted components or residue) or by in situ micro-mechanical mapping using techniques such as scanning acoustic microscope (SAM), atomic force microscope (AFM) respectively and nanoindentation.
Macroscopically, age-related differential dilatation of the intimal and medial layers is linked to whole vessel hypertrophy and dilatation12–16 whilst microscopically migration and cytoskeletal remodelling of VSMCs17,18 and fenestration/fragmentation of medial elastic lamellae17,19 have all been reported in the ageing artery. These architectural remodelling events may be accompanied and driven by age-related changes in vessel composition (primarily elastin and collagens I and III18–22) and molecular structure (via glycation, calcification, proteolytic degradation and fracture fatigue of long-lived ECM components15,23–29). To date, in part as a consequence of the historical methodological limitations inherent in measuring the mechanical properties of complex, heterogeneous materials such as large arteries, it has proven necessary to infer a causal relationship between gross tissue stiffening (as measured by in vivo or ex vivo techniques such as pulse wave velocity or tensile testing; for a detailed review see Ref.30) and the multiplicity of structural remodelling events which characterise the ageing artery. In the following section this review considers the contribution which new micro-mechanical approaches could make to identifying the key targets of arteriosclerosis by localising in situ mechanical changes to specific molecular components and/or tissue regions.
Micro-mechanical characterisation of arterial components
Three contrasting approaches have been used to characterise the mechanical properties of discrete arterial components and regions (Fig. 1B). First, chemical treatments such as concentrated formic acid or sodium hydroxide can be employed to differentially extract the majority of constituents from arterial walls prior to in situ mechanical testing of the remaining purified material.31 Even when suitably efficient however, the harsh chemical environments used by these differential extraction methods have the potential to profoundly disrupt the structure and hence mechanical properties of complex, multi-component fibrillar collagens and elastic fibres.2,32,33 A second approach is to extract and mechanically test specific arterial components, such as cells, in vitro. For example, in ground-breaking studies, Qiu and colleagues have demonstrated that the stiffness of cultured aortic VSMCs is related to age of the donor animal.1 However, the influence of in vitro culture conditions on cellular phenotype34 could be a key consideration in such studies. Finally the third approach, as adopted by our group, relies on technological advances to facilitate the in situ measurement of native mechanical properties at microscopical lengths scales (three complimentary technologies are summarised in Table 1). In turn these micro-mechanical measurements can be directly related to tissue architecture and composition with the ultimate goal of identifying key targets of age-related arteriosclerosis.
Nanoindenter | Scanning acoustic microscope (SAM) | Atomic force microscope (AFM) | |
---|---|---|---|
Lateral resolution (biological targets) | >10 μm (tissue regions) | 1 μm (cells/large ECM assemblies) | <10 nm (cellular components/small ECM assemblies) |
Depth resolution | Surface (nm to μm) | Specimen thickness (up to ∼6 μm for cryosections) | Surface (nm) |
Specimen interaction | Probably destructive | Non-destructive | Probably non-destructive |
Mechanical information | Multiple material characteristics (quantitative) | Acoustic wave speed (surrogate measure for stiffness) | Multiple material characteristics (may be qualitative or quantitative) |
Speed/sampling frequency | Slow sampling at discrete points | Rapid acquisition of acoustic images | Slow acquisition of mechanical maps |
Correlation with sample structure | Limited to post-test for conventional instruments | Acoustic images and histological images can be readily registered | Mechanical data can be registered with topographical (AFM) or histological data |
Availability | Readily available in Materials Science facilities | Limited availability | Readily available in multiple disciplines (including biomedical facilities) |
The in situ mechanical properties of tissues can be measured by three complimentary techniques. Nanoindenters, which are well established in engineering disciplines, have the capability to accurately measure the mean mechanical surface properties within a tissue region. In contrast, SAM can rapidly and non-destructively map acoustic wave speed maps (where wave speed is related to stiffness) at a resolution approaching 1 μm. In turn, these maps can be readily related to tissue structure as assessed by conventional histological approaches. Finally, the widely adopted technique of AFM is capable of mapping relative material characteristics and surface topography at sub-cellular and molecular length scale.
Physical indentation: nanoindenters and atomic force microscopes
Nanoindentation, which is the most common method for measuring the mechanical properties of a material at microscopical resolutions, employs a hard probe (of known mechanical properties) to physically indent a softer material (of unknown stiffness and/or resilience). Although originally developed to characterise engineering materials, in recent years the approach has been applied to both calcified35 and non-calcified biological tissues.30,36 Interpretation of the resultant load–displacement curves however, is complicated by the difficulties inherent in detecting the surface of highly compliant materials (including non-calcified biological tissue) and by the need to account for tip shape and surface roughness.30 In addition to these analytical difficulties, which must be overcome when indenting bulk tissues, further complications ensue when attempting to identify key mediators of tissue mechanical properties by co-localising nanoindentation and structural (histological) data in thin tissue sections. Whilst it is possible to avoid chemical fixation-induced stiffening37 by freezing and sectioning tissues (cryo-sectioning), interpretation of nanoindentation data obtained from such thin sections (commonly 5–10 μm thick) must take into account the influence of hard supporting substrates on the resultant displacement curves.36,38,39 Despite these analytical and practical difficulties nanoindentation approaches have been successfully employed to characterise the mechanical properties of soft tissues and materials (including viscoelastic or time dependent properties40) at μm to nm length scales.
There are two major classes of nanoindentation equipment: nanoindenters and atomic force microscopes (AFMs). In general nanoindenter employ a relatively large physical probe to accurately assess the mechanical properties of materials over μm length scales. Whilst the use of such large (up to 100 μm) diameter flat punch or conospherical probes aids in the detection of highly compliant biological surfaces this increase in sensitivity is achieved at the expense of spatial resolution.41 Hence the technique is best suited to measuring the mechanical contributions of discrete tissue regions rather than individual components. Despite these limitations we have successfully demonstrated the ability of a conventional nanoindenter (Hysitron Triboscope) to distinguish differences in substrate de-convolved tissue micro-stiffness between arteries and veins (aorta and vena cava) and within a vessel (from intimal to advential layer) at a spatial resolution of 25 μm.42 In common with other nanoindenter however (which are designed primarily for the characterisation of homogeneous engineering materials), this instrument lacks the optical capabilities to accurately direct nanoindentation of specific regions or components (such as the medial elastic lamellae). As a consequence we have collaborated with the same manufacturer (Hysitron Corp.) to specify the design of a bespoke instrument which will incorporate a nanoindenter head into the objective turret of a research quality optical microscope. It is envisaged that this instrument will facilitate the targeted indentation of tissue regions, elastic fibres and collagen fibrils (identified by differential interference contrast, circular polarised and auto-fluorescence microscopy respectively) in unfixed and unstained and cryosections. Following mechanical characterisation; tissue architecture and composition may be further characterised by conventional histological and immunohistochemical approaches.
As valuable as this new approach to biological nanoindentation promises to be, the ability of the technique to resolve fine-biomechanical detail will remain limited by the resolution of the probe. In order to quantify the in situ mechanical contribution of individual macro-molecular assemblies such as collagen fibrils43,44 or cells45 it will be necessary to use fine AFM probes. The ability of AFMs to combine high resolution mapping of both surface shape (topography) and mechanical properties has ensured that these instruments have been widely employed to characterise tissues, cells and biomaterials. Concerns exist however as to: (i) the applicability of conventional Hertzian mechanics approaches to the interpretation of AFM indentation data for high compliance materials,46 (ii) the difficulty in determining the spring constant of the cantilever on which the AFM probe is mounted47 and (iii) the influence of substrate mechanical properties on the measured sample properties.48 Although it may be argued that AFM-derived mechanical mapping data are best viewed as indicative of qualitative mechanical differences only49 a recent comparative study demonstrates that the Young’s moduli of bulk polymers (as measured by both AFM and conventional nanoindentation) are actually in good agreement.50
Non-invasive methods: scanning acoustic microscopy
As informative as nanoindentation approaches can be, the act of mechanically probing a material is: (i) slow (ranging from many minutes to many hours depending on sampling frequency/area and loading rate), (ii) limited to the vicinity of the surface (in contrast to structural information derived by light microscopy) and (iii) potentially damaging (particularly in the case of less compliant samples). Scanning acoustic microscopy (SAM) circumvents these difficulties by employing a pulse of high frequency waves (>100 MHz) to rapidly (in seconds) and non-destructively map local sample wave speed (which in turn is related to stiffness) throughout the specimen depth.30,48,51,52 Although well established in non-biological disciplines,53 the application of SAM to tissue sections has been limited and often applied to chemically fixed tissues54,55 or at lower frequencies and hence spatial resolutions.56 By employing cryosections and using frequencies close to ∼1 GHz however, it is possible to avoid fixation induced changes in the mechanical properties of tissues and to achieve spatial resolutions of ∼1 μm.
Such resolutions are required to resolve key arterial components and we have successfully employed SAM to localise the micro-mechanical manifestations of arteriosclerosis57 in an established model of sheep ageing.58 After initially demonstrating that, in common with rats and humans,59,60 sheep aorta undergo gross-mechanical stiffening, we then employed SAM to show that mean acoustic wave speed was also increased in the aged ovine aorta. Crucially however, this wave speed increase was both localised within the medial layer (to regions between elastic lamellae) and correlated with an increase in the collagen to elastin ratio. As informative as this approach proved to be, the structural resolution which was achievable after processing SAM data by the established frequency scanning method,52,61 made it difficult to interpret the apparent tissue architecture. Hence, we have recently presented an alternative method of SAM image collection and data processing (multi-layer phase analysis49) which employs phase differences to resolve and measure wave speed. The reduced signal noise in the resultant images greatly simplifies the task of identifying μm-scale tissue structures.
Selective oxidation as a potential causative mechanism of arteriosclerosis
Whilst in situ micro-mechanical testing approaches have the potential to identify the key targets of age-related arterial stiffening, other methodologies are required to isolate the causative molecular mechanisms. The ubiquitous nature of age-related tissue stiffening3 suggests that systemic mechanisms, as outlined in the introduction, may operate to promote cross-tissue ECM remodelling in mammals. One such mechanism however, the oxidative damage theory of ageing, has recently come under criticism, in part because the experimental evidence from short lived animal models such as fruit flies, nematode worms and mice indicates that attempts to shorten or prolong lifespan by increasing oxidative stress and enhancing antioxidant defences respectively are unlikely to meet with success.62,63 But in contrast to the environment within these relatively short-lived organisms (∼1 month lifespan for both Drosophila melanogaster and Caenorhabditis elegans), major ECM proteins including fibrillar collagens, elastin and fibrillin microfibrils are required to persist in human tissues for many decades64,65 where they must operate without the benefit of the damage prevention, detection and repair mechanisms which protect intracellular proteins (for reviews see Refs.3,66). As a consequence, we have suggested that reactive oxygen species (ROS) may play a key role in mediating the accumulation of age-related damage in ECM-rich human tissues such as skin, lungs and arteries.66,67 Drawing on both experimental evidence68 and bioinformatic analyses of relative amino acid compositions,67 we have proposed that elastic fibre associated proteins such as the fibrillins, fibulins and latent transforming growth factor β binding proteins (LTBPs) (which are rich in ultraviolet radiation [UVR] and ROS susceptible disulphide bonded cysteines) are preferentially denatured by UVR and/or ROS in vivo. In this scenario, ultrastructural modification of fibrillin-rich microfibrils (which is known to cause Marfan Syndrome in humans and mice) may be an important trigger event leading to loss of tissue homeostasis via aberrant TGFβ signalling/fibrosis69,70 and the upregulation of ECM-degrading matrix metalloproteinases.71 In contrast, the major structural ECM proteins collagen I and tropoelastin, which are largely devoid of UVR-absorbing and oxidation sensitive amino acids, are insensitive to physiological doses of both UVB and UVA radiation.68,72
Summary
Modifications of structural proteins play a key role in many age-related arterial disorders. Whilst our knowledge of the key mechanical targets and causative mechanisms is, as yet, limited the advent of new microscopical techniques brings an opportunity to further elucidate the pathological pathways. In addition there is much scope to draw on the techniques and knowledge accrued in biogerontological research to the specific case of the ageing artery.
Acknowledgements
I wish to acknowledge the great debt which I owe to my colleagues in the School of Materials (Prof. Brian Derby, Dr Riaz Akhtar [now at the Univ. of Liverpool], Dr Xuegen Zhao and Dr Craig Williams) and the Institute for Inflammation and Repair (Dr Helen Graham and Dr James McConnell) for their invaluable insight into the mechanical characterisation of tissues and the concomitant assessment of tissue structure. I would also like to thank my colleagues (Prof. Christopher Griffiths, Dr Rachel Watson and Dr Neil Gibbs) and students (Miss Sarah Thurstan and Miss Elizabeth Naylor) for their assistance with the oxidation studies referred to in this review.
References
Cite this article
TY - JOUR AU - Michael J. Sherratt PY - 2012 DA - 2012/12/29 TI - Structural proteins and arterial ageing JO - Artery Research SP - 15 EP - 21 VL - 7 IS - 1 SN - 1876-4401 UR - https://doi.org/10.1016/j.artres.2012.12.001 DO - 10.1016/j.artres.2012.12.001 ID - Sherratt2012 ER -