Predicting Immuno-Metabolic Complications After Allogeneic Hematopoietic Cell Transplant with the Cytokine Interleukin-33 (IL-33) and its Receptor Serum-Stimulation 2 (ST2)
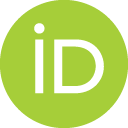
- DOI
- 10.2991/chi.d.200506.002How to use a DOI?
- Keywords
- ST2; IL-33; PTDM
- Abstract
Patients undergoing allogeneic hematopoietic cell transplantation (HCT) are at risk for numerous acute and long-term complications from this procedure. Post-transplant diabetes mellitus (PTDM) is a common but under-recognized problem. Similar to graft-versus-host disease (GVHD), new-onset diabetes is characterized by immune dysregulation that can negatively impact transplant outcomes. This review will discuss the biology of IL-33/ST2 in acute GVHD and PTDM development, and how this cytokine axis could be leveraged for predicting and treating immuno-metabolic complications after transplant.
- Copyright
- © 2020 International Academy for Clinical Hematology. Publishing services by Atlantis Press International B.V.
- Open Access
- This is an open access article distributed under the CC BY-NC 4.0 license (http://creativecommons.org/licenses/by-nc/4.0/).
1. INTRODUCTION
Allogeneic hematopoietic cell transplant (HCT) is an effective therapy for high-risk hematologic malignancies. It leverages the powerful graft-versus-leukemia (GVL) effect, in which alloreactive donor T lymphocytes recognize and eliminate malignant cells. Conversely, the major complication of HCT is graft-versus-host disease (GVHD), a process in which donor-derived T cells recognize normal organs as foreign, and initiate a complex cascade of humoral and cellular immune events, resulting in widespread recipient tissue damage [1]. Acute GVHD is observed in 30–60% of HCT recipients [2]. Patients with acute GVHD experience a significantly higher mortality rate compared to those without GVHD [3]. In the post-HCT period, previously euglycemic patients can also develop hyperglycemia related to insulin resistance and insulin insufficiency. Metabolic complications after HCT are common, with new-onset post-transplant diabetes mellitus (PTDM) developing in about 20–50% of patients, and generally preceding the diagnosis of acute GVHD. Similar to acute GVHD, PTDM is also associated with inferior overall survival (OS) following transplant [4,5].
GVHD and insulin resistance are both conditions that involve the accumulation of pro-inflammatory, type 1 T helper (Th1) cells that overwhelm the anti-inflammatory capacities of regulatory T cells (Tregs) and type 2 T helper (Th2) cells [6,7]. The cytokine interleukin-33 (IL-33), an alarmin, and its receptor serum-stimulation 2 (ST2) are involved in many inflammatory and autoimmune diseases, including allergic and respiratory disorders, inflammatory bowel disease, and rheumatoid arthritis. In HCT, soluble ST2 (sST2), a decoy receptor for IL-33, is a highly validated biomarker of steroid-refractory acute GVHD [8–11]. Diabetes and obesity are associated with low-level inflammation of adipose tissue. Interestingly, animal models of metabolic diseases demonstrate decreased levels of Tregs in the visceral adipose tissue (VAT), and the administration of IL-33 expands VAT Tregs and improves insulin sensitivity in these models [12,13]. This paper will review acute GVHD and PTDM in the context of immune dysregulation, the biology of the IL-33/ST2 axis as it relates to these disorders, and the role of plasma sST2 as a predictor of immuno-metabolic complications after HCT.
2. ACUTE GVHD
Acute GVHD usually occurs in the first 100 days after transplant, and is classically divided into three phases: recipient conditioning, donor T cell activation, and inflammatory effector [14]. First, pre-HCT conditioning regimens—incorporating radiation, chemotherapy, and depleting antibodies—damage and activate host tissues. Within this pro-inflammatory milieu, antigen presenting cells (APCs) upregulate MHC expression and lead to the next stage of acute GVHD. The second phase involves donor T-cell activation within secondary lymphoid organs. Allogeneic T cells expressing a suitable T-cell receptor interact with recipient APCs which express host major or minor histocompatibility antigen peptides. The activation of donor T cells leads to their proliferation and differentiation into Th1 and type 17 (Th17) helper T cells. Conversely, the activation of a subset of CD4+ Foxp3+ Tregs is able to suppress T-cell expansion and alloreactivity [15].
The third step, or effector phase, involves the migration of activated T cells from lymph nodes and spleen to GVHD target organs. Tissue destruction by alloreactive T cells occurs through the release of cytolytic molecules leading to donor cell apoptosis, cytolysis, cytokine release, and the recruitment of inflammatory cells. Immune injury within the target tissues of the skin, gut, or liver leads to the stereotypical symptomatology of acute GVHD: rash, nausea, diarrhea, and cholestasis. Tissue damage during either recipient conditioning or from the effector phase of acute GVHD results in the release of IL-33, which can then modulate cellular immunity, by binding to its receptor ST2 on T-cell subsets.
3. BIOLOGY OF IL-33 AND ST2
IL-33 is a member of the IL-1 superfamily of cytokines [16]. It is involved in the stimulation of integrin expression on leukocytes and endothelial cells, as well as in the initiation of inflammatory or immune-regulatory responses. It has a key role in both innate and adaptive immunity. Historically, IL-33 has been described as a potent inducer of allergic Th2 responses [17]. Recent research has identified a much broader functionality, with additional roles for IL-33 in tissue injury and repair, basal tissue regulation, as well as immunity to viruses and other microbes [17].
At steady state, the human IL-33 gene is constitutively expressed in the nuclei of multiple nonhematopoietic cell types, including endothelial, epithelial, and fibroblast-like cells [18,19]. The IL-33 protein can be found in almost all human organs, due to widespread endothelial expression along the vascular tree [18,20]. Epithelial cells in barrier tissues exposed to the environment, fibroblastic reticular cells in lymphoid organs, and central nervous system glial cells are major sources of IL-33 [18,19,21,22]. Furthermore, IL-33 expression can be increased during inflammation: increased levels of nuclear IL-33 are observed in the intestinal epithelium of HCT recipients with GVHD, in the airway epithelium of patients with chronic obstructive pulmonary disease, and in skin keratinocytes of patients with atopic dermatitis [23–25].
Unlike conventional cytokines, IL-33 lacks a signal sequence and is not secreted into the extracellular space [16]. Instead, it functions as a nuclear alarmin, an endogenous danger signal that is released after cell injury in order to alert the immune system of tissue injury during trauma or infection. IL-33 signals through a heterodimer complex consisting of its receptor ST2, and a shared signaling chain with IL-1 receptor accessory protein (IL1RAP) [26,27]. After engagement by its ligand, this complex recruits the Toll/IL-1 Receptor (TIR) domain binding protein MyD88, leading to IL-1R-associated kinase (IRAK) activation, with subsequent activation of mitogen-activated protein kinases (MAPKs) and nuclear factor-kβ (NFkβ) [28]. ST2 exists in two forms: a membrane-bound receptor and a soluble secreted protein (sST2). The membrane-bound receptor is widely expressed on a variety of immune cells, including Th2 cells, Tregs, group 2 innate lymphoid cells (ILC2s), M2-polarized macrophages, mast cells, eosinophils, basophils, neutrophils, NK cells, and inducible NK T cells [29–36]. sST2 functions as a decoy receptor that sequesters free IL-33 and prevents its signaling. Elevated sST2 levels are seen in GVHD, inflammatory bowel disease, pulmonary fibrosis, coronary artery disease, allograft rejection, and many other inflammatory conditions where it can modulate IL-33’s effects on immune cells [8,37–40].
By binding to its membrane-bound receptor ST2, IL-33 enhances the differentiation of diverse T cell subsets, improves T cell functionality, increases clonal expansion, and triggers antigen-independent cytokine production [41]. Its activity extends beyond Th2 cells and includes CD8+ cytotoxic T lymphocytes (CTLs), Th1 cells, and Tregs [41]. CTLs and Th1 cells often only transiently express ST2 during peak inflammatory conditions, and to a lesser extent than Tregs and Th2 cells, in which ST2 expression is constitutive [42]. This differential expression of ST2 is a mechanism for immune homeostasis, in that different T-cell subsets must compete for available IL-33 [41]. In homeostatic, noninflammatory conditions, Tregs and Th2 cells can sequester IL-33 from Th1 cells and CTLs, thereby limiting downstream inflammatory effects of ST2 signaling in the latter subsets [41]. During times of infection or significant tissue damage, necrotic cell death causes the release of sufficiently abundant quantities of IL-33 to also activate ST2+ Th1 cells and ST2+ CTLs, leading to proinflammatory effects. Thus IL-33 can have activating or regulatory effects on the immune system.
4. IL-33/ST2 SIGNALING AXIS AND PRECLINCAL MODELS OF GVHD
Mouse models of HCT and GVHD illustrate the pleiotropic nature of the IL-33 and ST2 signaling axis. Pre-HCT conditioning regimens increase endogenous IL-33 production by nonhematopoietic cells. IL-33 levels were shown to be significantly increased 24 and 48 hours after lethal total body irradiation (TBI), as well as chemotherapy-based conditioning regimens [23]. As per immunohistochemical staining of the small bowel in HCT recipients, IL-33 localizes to nonhematopoietic, CD45- cells. The transplantation of wild-type donor bone marrow and T cells into IL-33−/− recipients led to significantly reduced GVHD mortality and lower levels of pro-inflammatory cytokine TNF-α (compared to the magnitude of those effects in wild-type recipients). Conversely, the transplantation of IL-33−/− donor bone marrow and IL-33−/− T cells into wild type (WT) recipient mice failed to confer protection from GVHD. These data suggest that radiation-resistant, nonhematopoietic cells are the principal sources of IL-33 in the post-HCT period [23].
Compared to animals without GVHD (recipients of T cell-depleted allogeneic bone marrow alone), mice with GVHD (recipients of allogeneic bone marrow and allogeneic T cells) demonstrated significantly increased levels of sST2, which suggests that sST2 could serve to modulate GVHD severity [43]. In consideration of sST2’s function as a decoy receptor that sequesters IL-33 and prevents its signaling, Reichenbach and colleagues administered an exogenous sST2-Fc fusion protein, known to block IL-33 and membrane-bound ST2 interactions, and evaluated clinical and histopathologic GVHD severity [23]. Animals that received the fusion protein had significantly ameliorated GVHD compared to those that received only vehicle [23]. These data suggest that IL-33 is released by nonhematopoietic cells after tissue injury caused by pre-HCT conditioning regimens, and it exacerbates GVHD severity through the activation of donor ST2+ effector T cells. sST2 serves as a decoy receptor that sequesters free IL-33 and, as such, the exogenous administration of an sST2-Fc fusion protein that sequesters IL-33 can ameliorate GVHD in some preclinical models.
Similar to how heightened production of IL-33 in the post-HCT period leads to worse GVHD outcomes, the exogenous administration of IL-33 in the post-HCT period increases GVHD morbidity and mortality in murine models [23]. Recipients of post-HCT IL-33 showed expansion of the IFN-γ CD8+ donor T-cell compartment and increased levels of TNF-α compared to HCT controls that received vehicle only. Compared to HCT recipients of wild-type bone marrow and T cells, the adoptive transfer of wild-type bone marrow and ST2−/− T cells led to significantly reduced GVHD morbidity and mortality. These data seem to indicate that excess IL-33 signaling or lack of sequestration by sST2 is detrimental after HCT.
IL-33 functions as a dual cytokine depending on the inflammatory milieu and cell type expressing ST2. Accordingly, other data suggest that IL-33 can be anti-inflammatory during transplantation. In heart transplant models, IL-33 administration prolongs cardiac allograft survival through Treg expansion and the induction of Th2 cell responses [44]. Intriguingly, though post-HCT IL-33 administration exacerbated GVHD, giving IL-33 before HCT conditioning led to the development and expansion of ST2+ Tregs, which significantly ameliorated GVHD severity [45]. In contrast, the selective depletion of Foxp3+ cells concurrent with peri-HCT IL-33 administration accelerated GVHD lethality [45]. IL-33-expanded Tregs limit GVHD severity through prevention of target organ accumulation of alloreactive effector T cells, as well as by controlling macrophage activation. The adoptive transfer of ST2+, but not ST2-, Tregs decreased alloreactivity. These data suggest that IL-33’s effects on differing T cell subsets could mediate its pro- and anti-inflammatory effects [23,45]. IL-33 and ST2 appear to play a major role in modulating acute GVHD severity and, thus, it would seem logical that this cytokine axis could be leveraged for predicting and treating alloreactivity. Given the cytokine’s pleomorphic nature, further research is needed to better define the role of IL-33/ST2 axis in acute GVHD.
5. sST2 AS A PROGNOSTIC MARKER FOR CLINICAL ACUTE GVHD
Although mortality after HCT has improved over the past six decades, acute GVHD remains a significant complication that occurs in approximately half of HCT recipients [46]. Clinical risk factors for GVHD include the degree of human leukocyte antigen (HLA) match between donor and recipient, recipient age, donor type, stem cell source, and conditioning regimen intensity [47,48]. For the past 40 years, the primary treatment for acute GVHD has been high-dose systemic glucocorticoids, but only half of patients with GVHD achieve complete resolution by day 28 after corticosteroid initiation [2]. Steroid-refractory GVHD patients are at a high risk for nonrelapse mortality (NRM), with rates as high as 70–90% [47–52]. The inability to risk-stratify individuals at symptom onset often leads to either overtreatment or undertreatment, and it presents an important obstacle to the development of new therapies for GVHD. As most patients are treated similarly with corticosteroids, iatrogenic complications such as infection, myopathy, hyperglycemia, and avascular necrosis of bone are common [48,53,54]. As such, there is a significant need for biomarkers that can predict and prognosticate acute GVHD.
Independently of the previously described animal models, Paczesny and colleagues were the first to identify plasma sST2 as an effective marker to predict treatment-refractory GVHD and NRM in human transplantation (Table 1). Plasma samples were obtained from 20 patients with acute GVHD— ten with a complete response to GVHD therapy and ten with refractory disease [8]. Six plasma markers: sST2, macrophage inhibitory factor, interleukin-1 receptor, lipocalin 2, lymphatic-vessel endothelial hyaluronan receptor 1, and regenerating islet-derived protein 3-α (REG3α), were found to be significantly elevated in refractory GVHD as compared to those with complete responses to treatment [8]. Of these, sST2 had the strongest association with nonresponse to therapy and NRM. To determine its association with treatment-resistant GVHD and subsequent 6-month NRM, sST2 was prospectively measured from the plasma samples of 381 patients at the initiation of therapy for GVHD and 14 days after transplantation. As compared with patients with low sST2 values (sST2 < 740 picograms/mL), patients with high values (sST2 ≥ 740 picograms/mL) were 2.3 times as likely to have treatment-resistant GVHD (95% CI, 1.5–3.6) and 3.7 times as likely to die within 6 months of therapy (95% CI, 2.3–5.9) [8]. Patients with low sST2 values had lower NRM than patients with high sST2 values, regardless of the GVHD grade [8]. sST2 levels measured at the initiation of therapy for GVHD and during the first month after transplantation improved risk stratification for treatment-resistant GVHD and death without relapse after transplantation [8].
Outcome | Biomarker | N | Date analyzed | Direction of response | References |
---|---|---|---|---|---|
Treatment-resistant GVHD, NRM | sST2 | 20 + 381 + 673 + 75 | Therapy initiation, D+14 | Increased | Vander Lugt et al. [8] |
Treatment-resistant GVHD, NRM | sST2, REG3α, TNFR1 | 328 + 164 + 300 | GVHD onset | Increased | Levine et al. [10] |
NRM | sST2, TNFR1 | 74 + 76 | GVHD onset | Increased | McDonald et al. [67] |
GVHD, NRM in CBT | sST2 | 113 | D+28 | Increased | Ponce et al. [9] |
GVHD, NRM | sST2, REG3α | 620 + 309 + 358 | D+7 | Increased | Hartwell et al. [11] |
GVHD, NRM | sST2, REG3α | 248 + 367 | Therapy initiation, 28 days after GVHD treatment | Increased | Srinagesh et al. [68] |
NRM | sST2, REG3α, TNFR1 | 170 + 245 | Pre-HCT, D+7, D+14, D+21 | Increased | Rowan et al. [69] |
Malignancy relapse, NRM | sST2,REG3α | 702 + 902 | D+28 | Relapse decreased, NRM increased | Aziz et al. [70] |
PTDM, NRM | sST2 | 36 + 26 | Engraftment, D+30 | Increased | Johnpulle et al. [60] |
PTDM, all-cause mortality | sST2 | 55 | D+14 | Increased | Rowan et al. [61] |
GVHD, graft-versus-host disease; sST2, soluble ST2; REG3α, regenerating islet-derived protein 3-α; TNFR1, tumor necrosis factor receptor 1; CBT, cord blood transplant; HCT, hematopoietic cell transplant; PTDM, post-transplant diabetes mellitus.
Biomarkers for immuno-metabolic complications after allogeneic hematopoitic cell transplant
In another study, Paczesny and Barker noted that sST2 was independently associated with acute GVHD after day +28 in cord blood transplantation (CBT) recipients [9]. At D+28, serum or plasma samples from 113 CBT patients (all of whom received double-unit CBTs) were analyzed for levels of markers including sST2, REG3α, interleukin2 receptor α (IL2Rα), tumor necrosis factor receptor 1 (TNFR1), elafin, hepatocyte growth factor (HGF), and interleukin-8 (IL-8). While TNFR1, REG3α, and IL-8 were associated with NRM, sST2 was the only biomarker associated with both acute GVHD and NRM. Stratified by high (>33.9 ng/mL) or low (≤ 33.9 ng/mL) sST2 levels, patients in the high group had significantly increased rates of grade III-IV acute GVHD and NRM by day +180 than did those in the low group [GVHD 30% versus. 13%; p = 0.024; NRM 23% versus. 5%; p = 0.001] [9].
To further refine meaningful risk strata, Levine and colleagues, evaluated three biomarkers in 492 HCT recipients with newly diagnosed acute GVHD in a multicenter study [10]. The three-biomarker panel, consisting of the previously validated proteins TNFR1, REG3α, and sST2, was used to compute the predicted probability of NRM within 6 months of GVHD diagnosis [10]. Plasma samples were obtained at the onset of acute GVHD. The authors identified biomarker thresholds and rank ordered them from lowest to highest to create three distinct NRM scores, known as the Ann Arbor GVHD score, such that a score of 1 corresponded to a risk of NRM ≤ 10% and a score of 3 to a risk of NRM ≥ 40%. In all three independent datasets (training, test, and validation) the cumulative incidence of 6-month NRM significantly increased and the response to primary GVHD treatment within 28 days decreased as the Ann Arbor GVHD score increased [10]. The authors concluded that biomarker-based scores can be used to guide risk-adapted therapy at the onset of acute GVHD, and suggested that high-risk patients with an Ann Arbor score of 3 are candidates for intensive primary therapy or novel agents, while low-risk patients with a score of 1 are candidates for rapid taper of systemic steroids [10].
Given the success of biomarker panels in risk-stratifying acute GVHD at symptom or treatment onset, Hartwell and colleagues sought to determine whether a biomarker signature before acute GVHD onset could be predictive of treatment response and NRM [11]. Blood samples on day +7 after HCT were obtained from a multicenter set of 1,287 patients, and four GVHD biomarkers, sST2, REG3α, TNFR1, and IL-2Rα, were measured to model 6-month NRM. The most accurate model included concentrations of sST2 and REG3α and identified high-risk and low-risk groups, with NRM of 28% and 7%, respectively (p < 0.001). The simplified two-protein biomarker set also correlated with the previously described Ann Arbor GVHD score. Independent of important clinical risk factors, such as the degree of HLA mismatch, the genetic relationship between donor and recipient, the intensity of conditioning regimen, and the age of the recipient, the biomarker algorithm was able to stratify patients into high or low risk for NRM [11]. While HLA mismatch and donor–recipient relationship were significantly associated with NRM in univariate analyses, their incorporation into the algorithm did not significantly improve its performance over the sST2-REG3α-biomarker panel alone. High-risk patients had a three-fold higher NRM risk than low-risk patients (NRM 19% versus 6%, respectively; p < 0.001) and experienced greater than twice the steroid-refractory GVHD as did low-risk patients (treatment refractory 35%, versus 15%; p < 0.001). Furthermore, high-risk patients experienced twice as much severe gastrointestinal GVHD as did low-risk patients [11]. The increase in REG3α and sST2 prior to onset of GVHD symptoms suggests the biomarkers can identify subclinical gastrointestinal pathology. These observations have led to biomarkers and the Ann Arbor scoring system being incorporated into clinical practice. Clinical trials using biomarker risk stratification at the time of GVHD onset followed by treatment with the novel agents itacitinib (JAK1 inhibitor) or natalizumab (α4 integrin blocker) for Ann Arbor scores 1 (low-risk) or 3 (high-risk), are currently underway (NCT03846479 and NCT02133924, respectively).
6. PTDM AFFECTS HCT OUTCOMES
As HCT survival increases, long-term care needs to focus on preventing or minimizing chronic treatment-related complications. Along with GVHD, the study of the metabolic complications after HCT is important due to their negative impact on cardiovascular health and survival. Compared with recipients of autologous HCT, recipients of allogeneic HCT are at increased risk for metabolic syndrome and PTDM. Approximately 20 to 50% of HCT recipients develop new-onset PTDM, and mortality for those with PTDM is three-fold higher than those without hyperglycemia [4,5]. Clinical risk factors for PTDM include TBI, increased body mass index, unrelated donor source, ablative conditioning, older age, and higher doses of corticosteroids [5,55]. However, the mechanistic causes for PTDM have not been well-described.
Griffith et al. conducted a prospective study of patients undergoing HCT with calcineurin-inhibitor-based GVHD prophylaxis to evaluate PTDM risk factors and to describe the incidence of new-onset PTDM [5]. Adult patients, without a prior diagnosis of type-2 diabetes mellitus and who were scheduled for their first HCT, were included. Baseline laboratory values, including fasting glucose, fasting lipid profile, C-peptide, and insulin, along with height, weight, and vital signs, were obtained one day prior to conditioning. The authors prospectively defined PTDM as a fasting blood glucose ≥ 126 mg/dL or a random blood glucose ≥ 200 mg/dL [5]. Weight and fasting glucose were measured weekly until either PTDM diagnosis or day +100 post-HCT.
Patients underwent either ablative or reduced-intensity (RIC) conditioning regimens. The GVHD prophylaxis incorporated calcineurin inhibitors and methotrexate (in ablative) or mycophenolate mofetil (in RIC). Acute GVHD was treated according to standardized institutional guidelines, with grade II-IV aGVHD treated with 1–2 mg/kg/day prednisone equivalent for 10–14 days, followed by a 10% weekly taper. The primary endpoint was PTDM incidence by day 100. Assessments of PTDM risk factors and survival were the secondary aims.
Eighty-four patients completed the study. The demographic data, BMI, blood pressure, category of malignancy did not differ between patients who did and did not develop PTDM. Fifty (60%) patients developed PTDM. The cumulative incidence of PTDM was 50% by day 41 and 59% by day 98. The median time from transplant to PTDM diagnosis was 23 days, and generally preceded GVHD diagnosis or corticosteroid treatment. Univariate risk-factor analysis showed an increased risk of PTDM in patients who received ablative conditioning, peak systemic steroids ≥ 1 mg/kg/day, unrelated donor HCT, or TBI. Pre-HCT fasting C-peptide levels were higher in patients with PTDM (4.45 ng/mL versus 2.6 ng/mL; p = 0.025) [5]. The incidence of PTDM was 72.5% in patients with a fasting, pre-transplant C-peptide above the cohort median of 3.6 ng/mL as compared with 50% of patients with C-peptide ≤ 3.6 ng/mL. Furthermore, in a multivariate logistic regression model, pre-HCT fasting C-peptide level >3.6 ng/mL (OR 5.9; 95% CI 1.77–20.44; p = 0.004), unrelated donor HCT (OR 4.3; 95% CI, 1.34–14.2; p = 0.014), and peak steroid use >1 mg/kg/day (OR 5.09; 95% CI, 1.19–23.2, p = 0.035) were independent predictors of PTDM development [5]. The mean survival of patients with PTDM was 2.26 years compared to 2.7 years for those without PTDM (p = 0.021). Analysis of PTDM as a time-dependent variable showed its significant impact on OS (HR 3.27; 95% CI, 1.3–8.2; p = 0.01). Not only did pretransplant, fasting C-peptide greater than the cohort median (>3.6 ng/mL) predict PTDM diagnosis, it was also associated with inferior OS (1.7 years versus 2.9 years, p = 0.012). Thus, a strong relationship between elevated fasting C-peptide levels and both PTDM and mortality was identified. In this study, the elevation of fasting C-peptide levels was thought to represent normal/physiologic β-cell compensation related to established insulin resistance. Taken together, these data suggest that insulin resistance precedes PTDM, and that factors associated with insulin resistance lead to inferior HCT outcomes. The fact that the elevations in C-peptide were noted before transplant means that these findings cannot be due to immunosuppression or alloreactivity.
Based on the C-peptide data, and to determine if PTDM was due to preexisting insulin resistance, Engelhardt and colleagues prospectively followed 20 patients,without diabetes, and who underwent related HLA-identical, peripheral blood HCT with either ablative or RIC conditioning, for the development of new-onset PTDM [56]. To measure whole-body, peripheral, and hepatic insulin sensitivity, the authors performed oral glucose tolerance testing (OGTT) and the gold-standard euglycemic hyperinsulinemic clamp on patients both before and 90 days after HCT [56]. In the first 100 days post-HCT, 55% of recipients developed PTDM (at a median of 22 days post-HCT), and PTDM diagnosis preceded both corticosteroid use and the development of grades II-IV acute GVHD. No patients were receiving corticosteroids at the time of PTDM diagnosis. During pre-HCT OGTT, elevated fasting blood glucose (87 mg/dL versus 101 mg/dL; p = 0.005), but not 2-hour post-prandial glucose level, predicted PTDM diagnosis [56]. When compared to patients who maintained euglycemia, HCT recipients who developed PTDM exhibited lower pre-HCT whole-body insulin sensitivity, as well as lower skeletal muscle glucose uptake post-HCT. There were no significant differences in hepatic glucose production or hepatic insulin sensitivity among patients with PTDM and euglycemic HCT recipients. The estimated two-year OS was decreased in patients developing PTDM compared to those who maintained euglycemia (55% versus 100%; p = 0.039) [56]. These data suggest that the risk of progression to PTDM depends on preexisting, subtle whole-body insulin resistance before HCT, which develops into more robust peripheral/skeletal muscle insulin resistance after HCT. These findings also firmly repudiate the misconception that PTDM is an iatrogenic phenomenon secondary to hyperglycemia-inducing immunosuppressive medications. Targeting insulin resistance or the immuno-metabolic consequences of pre-diabetes could be a rational approach for decreasing complications and improving HCT outcomes.
7. THE IL-33/ST2 AXIS IN OBESITY AND TYPE-2 DIABETES MELLITUS
The accumulation of VAT in obesity leads to chronic inflammation, insulin resistance, and the development of type-2 diabetes mellitus [57]. Immunologically, the progression of insulin resistance to diabetes mellitus is characterized by the accumulation of Th1 cells and depletion of immunosuppressive Tregs in adipose tissue, leading to low-grade systemic and localized inflammation [7,12]. The expansion of VAT Tregs through the administration of recombinant IL-33 led to lower adiposity, reduced fasting glucose, and improved glucose tolerance and insulin sensitivity in genetically obese mice [13]. IL-33 also induced the accumulation of Th2 cells in adipose tissue and polarization of adipose tissue macrophages toward an M2 alternatively activated phenotype, which is associated with protection against obesity-related metabolic events [58]. Conversely, ST2−/− mice not susceptible to the effects of IL-33 had increased body weight, abnormal glucose regulation, and impaired insulin secretion when compared to wild-type control animals fed a high-fat diet [58]. Taken together, the balance between adipose tissue inflammation versus homeostasis and the ratio Th1 cells to ST2+ VAT Tregs are critical determinants of metabolic regulation. In turn, the IL-33/ST2 signaling axis appears to be a major factor driving this equilibrium, which could be disturbed after HCT.
Corticosteroids are commonly used during HCT, and glucocorticoid-induced hyperglycemia is well known. However, there is a paucity of data describing the direct effects of glucocorticoids on the IL-33/ST2 signaling axis. In a mouse model of allergic airway disease, treatment with intranasal budesonide reduced inflammation but bronchoalveolar IL-33 levels remained unchanged [59]. More research will be needed to describe the effects of glucocorticoids on the IL-33/ST2 axis during HCT.
8. sST2 AS A BIOMARKER FOR PTDM
Evidence suggests that IL-33 regulates insulin sensitivity, established insulin resistance precedes PTDM, and PTDM leads to decreased survival after HCT. As discussed, sST2 is a validated biomarker for steroid-refractory acute GVHD and mortality after transplant. Therefore, Johnpulle and colleagues hypothesized that PTDM was related to dysregulated IL-33/ST2 signaling, and that sST2 would predict PTDM diagnosis [60]. Serum sST2 was measured at neutrophil engraftment and at day +30 in 36 euglycemic HCT patients, who were followed prospectively as above for the development of PTDM. An independent validation cohort of 26 patients without pre-HCT diabetes was analyzed retrospectively for PTDM diagnosis. Compared to patients without PTDM, patients with PTDM had higher levels of sST2 at engraftment (p = 0.02) and at D+30 (p < 0.01). Elevated sST2 levels among PTDM patients were confirmed in the second cohort at engraftment (p = 0.01) (Table 1). Multivariate analysis revealed that high engraftment sST2 levels continued to predict PTDM and NRM, independent of conditioning and GVHD. These data indicate a relationship between glucose homeostasis and the IL-33/ST2 axis after adult transplantation [60].
Pediatric HCT recipients also demonstrate a significant association between PTDM and sST2 [61]. At a different transplant center, 55 pediatric HCT patients without preexisting diabetes were followed for the first 100 days post-HCT. The authors monitored fasting and random blood glucose levels on a weekly basis and obtained plasma sST2 levels at day +14. PTDM was diagnosed in 31% of patients. The median time to PTDM diagnosis was day +19 (range 3 to 44 days). Between patients with and without PTDM, gender, age, malignancy, matched or related donor status, disease risk index, use of high-dose corticosteroids (≥ 1 mg/kg methylprednisolone equivalent for at least 72 hours), and BMI did not differ significantly. Patients who received umbilical CBT were more likely to develop PTDM (p = 0.0363). Patients with PTDM had worse HCT outcomes, including increased likelihood of admission to the intensive care unit (71% PTDM versus 8% without PTDM; p = 0.007) and greater risk for mechanical ventilation (53% PTDM versus 5% without PTDM), as well as decreased OS at one year (50% PTDM versus 90% without PTDM; p = 0.0239). Patients with PTDM also had higher mean sST2 levels at day +14 compared to patients without PTDM (sST2 95.1 ± 90.0 ng/mL versus 28.2 ± 28.7 ng/mL; p = 0.0002).
In a subsequent analysis, sST2 levels were categorized as either “high” or “low” using the median sST2 level of the cohort as the threshold [61]. Patients with a high sST2 level had increased risk of developing PTDM (HR 3.92; 95% CI 1.42–10.84; p = 0.0085). Interestingly, analysis of pre-HCT sST2 levels did not show significant differences between patients who developed PTDM and those who did not, although this comparison was limited by an incomplete dataset. Collectively, two HCT studies from different institutions involving adult or pediatric cohorts have now shown that PTDM is associated with decreased survival, and that high sST2 levels in the first 2–3 weeks of transplant predict PTDM development.
Although sST2 has been shown to be a validated predictor of glucose dysregulation, it remains unclear if this protein is the best biomarker for PTDM. Similarly to the identification of biomarkers of acute GVHD, proteomic and transcriptomic approaches will need to be applied to the study of metabolic complications to identify the best and most precise biomarkers for PTDM. Furthermore, additional studies in metabolic HCT outcomes are needed to determine the effects of corticosteroids and the IL-33/ST2 signaling axis. However, in the studies reviewed here, sST2 levels were typically analyzed before the development of PTDM and corticosteroid use and, so, steroid administration should not affect the initial values.
9. CONCLUSIONS/FUTURE DIRECTONS
GVHD and PTDM are common complications after HCT, which are characterized by immune dysregulation and inferior survival. The pleiomorphic cytokine IL-33, its receptor ST2, and sST2 are intricately linked to both conditions. In HCT, depending on the inflammatory milieu, IL-33 can have differing effects. During baseline homeostasis, the pre-HCT administration of IL-33 seems to expand ST2+ Tregs and dampen GVHD severity. However, in the setting of tissue damage, post-HCT administration of IL-33 can activate IFNγ-producing T cells that exacerbate GVHD [23,45]. Similar to alloreactivity, the development of insulin resistance and type-2 diabetes mellitus is associated with chronic inflammation and immune activation. Animal models of insulin resistance have demonstrated an accumulation of Th1 cells and depletion of ST2+ Tregs in adipose tissue [12,13,58]. Within these preclinical models, IL-33 administration results in VAT ST2+ Treg expansion and improvement in insulin sensitivity [13].
Given the role of IL-33/ST2 in regulating cellular immunity and insulin sensitivity, it is not surprising that the decoy receptor sST2 is a validated biomarker for acute GVHD, PTDM, and transplant mortality [8–11,60,61]. In addition, cardiovascular diseases such as coronary artery disease, heart failure, and stroke have also been linked to IL-33/ST2 signaling, and these complications are significant causes of morbidity and mortality for long-term survivors of HCT [62–66]. Thus, monitoring sST2 may have implications beyond just glucose metabolism and GVHD.
sST2 as well as other GVHD biomarkers are now being incorporated into therapeutic clinical trials. As our understanding of IL-33/ST2 increases, this cytokine axis could be leveraged for treating immuno-metabolic complications after transplant. For example, Zhang and colleagues demonstrated that by blocking sST2 with a monoclonal antibody, IL-33 levels can be increased in the peri-transplant period, leading to less severe GVHD and improved HCT outcomes in an animal model [43]. A similar strategy could be used to mitigate metabolic inflammation and insulin resistance. In conclusion, modulation of IL-33 signaling, heightened awareness of metabolic complications after HCT, and optimized treatment strategies for insulin resistance could all potentially improve transplant outcomes. This intriguing therapeutic possibility warrants the development of experimental animal models of PTDM, as well as randomized clinical trials for the treatment and prevention of metabolic complications after HCT.
CONFLICTS OF INTEREST
The authors declare they have no conflicts of interest.
AUTHORS' CONTRIBUTION
UKR and BGE both had equal contribution to the conception of the work, drafting the work, revising it and approval of the final manuscript.
ACKNOWLEDGMENTS
This work was supported by National Institutes of Health/National Heart, Lung, and Blood Institute Grant R01-HL141943 (to B.G.E) and K23-HL-122143-01A (to B.G.E.).
REFERENCES
Cite this article
TY - JOUR AU - Uttam K. Rao AU - Brian G. Engelhardt PY - 2020 DA - 2020/05/21 TI - Predicting Immuno-Metabolic Complications After Allogeneic Hematopoietic Cell Transplant with the Cytokine Interleukin-33 (IL-33) and its Receptor Serum-Stimulation 2 (ST2) JO - Clinical Hematology International SP - 101 EP - 108 VL - 2 IS - 3 SN - 2590-0048 UR - https://doi.org/10.2991/chi.d.200506.002 DO - 10.2991/chi.d.200506.002 ID - Rao2020 ER -